|
02 Features
Print To PDF
DisplayTitle Nutritional Genomics: Connecting Crop Improvement to Human Health
Affiliations Department of Agronomy, Kansas State University, Manhattan, KS, U.S.A.
Department of Agronomy, Kansas State University, 3108 Throckmorton Plant Sciences Center, 1712 Claflin Rd, Manhattan, KS 66506, U.S.A. Tel: +1.785.532.2166; E-mail: davinarhodes@ksu.edu
Abstract
CFWAbstract Staple cereal crops contribute the majority of calories in human diets worldwide. Wheat, rice, maize, pearl millet, and sorghum together provide 44% of all calories consumed per capita per day worldwide, so improving the nutritional quality of cereal grains could have a significant impact on alleviating malnutrition. The discipline of crop nutritional genomics will be a key contributor toward ensuring global food security through biofortification.
Trying to reach content?
View Full Article
if you don't have access, become a member
Page Content One in three people worldwide are malnourished (12). Malnutrition encompasses both undernutrition (too little energy, protein, or micronutrients in the diet) and overnutrition (too much energy in the diet), and these two forms of malnutrition increasingly coexist in many countries (12). Undernutrition is associated with increased risk of infectious disease, stunted growth, and severe wasting, whereas overnutrition is strongly associated with development of chronic diseases such as type 2 diabetes, cardiovascular disease, and some forms of cancer. Globally, 2 billion adults and 40 million children are overweight or obese, 200 million children are stunted or wasted, and 2 billion people are micronutrient deficient (12). The prevalence of malnutrition—exacerbated by a ballooning world population that is estimated to reach nearly 10 billion by 2050 and shifts in agroecological zones due to climate change—is an increasingly urgent threat to food security. The world needs to produce more food and more nutritious food at a faster rate than ever before.
Staple cereal crops contribute the majority of calories in human diets worldwide. Wheat, rice, maize, pearl millet, and sorghum together provide 44% of all calories consumed per capita per day worldwide, so improving the nutritional quality of cereal grains could have a significant impact on alleviating malnutrition (16). As crop yields have been pushed higher over the last 30 years, hunger has decreased significantly, falling by nearly 40% since 1990, but malnutrition is still prevalent (39). This is explained, in part, by the fact that we have been able to grow more food crops, but not food crops that are more nutritious. Crop improvement efforts tend to focus on increasing yield and agronomic stability, rather than nutritional quality, partly due to the challenges involved in integrating human nutrition research (6).
Nutritional Genomics and Biofortification
The discipline of crop nutritional genomics will be a key contributor toward ensuring global food security through biofortification. In crop science, nutritional genomics can be defined as the study of how the genome of a plant affects the nutrient composition of the plant and how, in turn, plant nutrient composition impacts human nutrition. Through the integration of crop genomics, biochemistry, and nutrition, knowledge generated by nutritional genomics research is used to inform efforts to enhance the nutritional value of food crops to improve human nutrition and health (11).
The process of developing nutrient-dense food crops through breeding, biotechnology, or agronomy is termed biofortification. Biofortification is used most often in reference to enrichment of micronutrients (vitamins and minerals), but the term can be used to describe the enrichment of any nutrient in a food crop (36). Through biofortification, a crop that typically produces low concentrations of a nutrient gains the biosynthetic capacity to produce nutrients in sufficient amounts to have a beneficial impact on human or animal health. Biofortification complements other strategies for improving nutritional status, including dietary diversification (consumption of a variety of foods), supplementation (intake of a manufactured nutrient), and fortification (addition of a nutrient to a food during processing), but when compared with these strategies, biofortification is more cost-effective, sustainable, and accessible (6).
Breeding and Biotechnology
Crop biofortification efforts over the last couple of decades have been highly successful, due in part to advances in breeding and technology that have enabled rapid progress in nutritional genomics (2,5,9,23,44). Millions of people worldwide grow and consume hundreds of biofortified crop varieties, including high-provitamin A maize in Zambia, high-iron pearl millet in India, and high-zinc rice and wheat in Bangladesh and Pakistan (6).
A workflow for nutritional genomics research is illustrated in Figure 1. This workflow emphasizes the need for an integrative approach to the process. The first step in nutritional genomics research is to characterize variation in concentrations of the nutrient of interest within the crop of interest. Nearly 2,000 crop genebanks are used to store millions of crop varieties around the world and are dedicated to protecting food security through preservation of crop genetic diversity (15). Cereal crops—predominantly wheat, rice, barley, maize, sorghum, and pearl millet—make up approximately 45% of all the holdings in crop genebanks worldwide (15). These holdings are freely available for research and are a crucial resource for finding new sources of nutrient diversity.
When variation in a nutrient is found, varieties with high concentrations of the nutrient can be used to introgress favorable alleles that will confer high nutrient concentrations into elite breeding varieties. Introgression is the process of moving alleles from one variety to another through traditional plant breeding (Fig. 2). Because a variety with a high nutrient concentration often does not have many other agronomically acceptable traits for the geographic region where it will be grown (e.g., drought or disease resistance) or the people who will consume it (e.g., flavor or starch content), favorable alleles need to be introgressed into a locally adapted and consumer preferred variety.
Measuring the nutrient concentrations in thousands of varieties held in a crop genebank to identify which varieties to use for introgression is both cost- and time-prohibitive. Marker-assisted selection—use of genetic markers to select varieties containing favorable alleles that confer high nutrient concentrations—can accelerate the breeding process. To enable marker-assisted selection of nutrients, hundreds of varieties that vary in nutrient concentrations are genotyped to identify genetic markers that control nutrient variation among varieties (27,32,33). Scanning the genome for statistical associations between the genotypes and phenotypes of each variety, using genome-wide association studies or linkage studies, regions in the genome (markers) that control nutrient concentrations can be identified. The genetic markers can then be used by breeders to rapidly identify which seedlings will eventually produce high concentrations of the nutrient in the grain without having to wait for the seedling to mature and produce grain.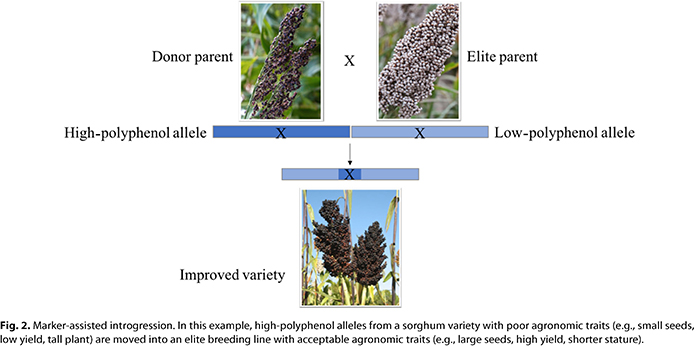
If little or no variation is found, then breeding will not significantly increase nutrient concentrations, and scientists have to turn to biotechnology for biofortification solutions. This can be done by transferring genes from one species to another, by inducing mutations, or through gene editing. However, public anxiety over crops developed through biotechnology, despite decades of scientific evidence pointing to their safety, has prevented broad acceptance of their consumption (35).
Another crucial step in nutritional genomics research is to characterize the bioavailability and health benefits of the biofortified crop. Bioavailability refers to the proportion of the nutrient that is available for use in the body after digestion and absorption. Nutrient bioavailability depends on many factors, including crop variety, the composition of the food eaten with the biofortified crop, and the health status of the person consuming the food. Cell, animal, and human studies are conducted to determine the bioavailability and health benefits of a nutrient, which, in turn, help to determine the target concentration of a nutrient for biofortification.
Crop varieties that have the most potential are grown and studied by breeders in multiple locations over multiple years and, if the crop trials are successful, eventually are released to farmers. These workflow steps are not carried out linearly, but are instead usually conducted in parallel or in cycles before the final biofortified product is released. In this way, by combining the tools of nutritional genomics (biochemistry, crop genomics, and human nutrition) germplasm can be characterized relatively quickly and cost-effectively for diversity, genetic controls, and health benefits and selected for use in biofortification.
In the following sections, nutritional genomics is illustrated through three examples of efforts to biofortify major staple cereals. These examples demonstrate how nutritional genomics research has successfully informed the process of biofortification.
Examples of Biofortification of Cereal Crops
Golden Rice. In the early 1990s, scientists Ingo Potrykus and Peter Beyer embarked on a project that would eventually lead them to fame and controversy. Their goal was to biofortify rice with provitamin A carotenoids in order to save millions of children from blindness, infections, and death due to vitamin A deficiency. Carotenoids are a large group of yellow, orange, and red pigments (e.g., orange b-carotene in carrots and red lycopene in watermelon) that are made by all photosynthetic organisms. Some types of dietary carotenoids act as antioxidants and anti-inflammatories in the body, while others, such as b-carotene, are converted to vitamin A in the body (provitamin A carotenoids).
Vitamin A deficiency is one of the most prevalent micronutrient deficiencies worldwide, affecting 190 million preschool-age children and 10 million pregnant women, predominantly in South Asia and sub-Saharan Africa (40). It is the leading cause of preventable blindness in children under 5 years of age and contributes to thousands of early childhood deaths due to diarrhea, measles, malaria, and intestinal parasites. The primary underlying cause of vitamin A deficiency is low dietary intake of carotenoids. Cereal grains, which are staples in regions with high vitamin A deficiency, are generally poor sources of carotenoids, so efforts to combat dietary insufficiency through biofortification of staple crops could have a significant impact on reducing vitamin A deficiency.
Rice is a staple crop for more than half of the global population, particularly in Asia, providing 60–80% of daily caloric intake (16). Rice endosperm, the starchy part of the grain that is consumed in the diet, is not able to naturally synthesize carotenoids, because part of the carotenoid biosynthetic pathway is “turned off.” Knowing there was no carotenoid variation in the rice endosperm, Potrykus and Beyer worked to find a transgenic “on switch” for the carotenoid biosynthetic pathway. Transgenic crops had been a subject of heated debate and protest since the 1980s (Potrykus grew his transgenic plants in a hand grenade-proof greenhouse), but with no breeding option, they forged forward to develop a transgenic solution (31).
The first experiments revealed that a key enzyme, phytoene synthase, was missing in the rice endosperm. This enzyme catalyzes the formation of phytoene, the first carotenoid in the biosynthetic pathway, which is necessary for formation of b-carotene. To fix this part of the biosynthetic pathway, they inserted a daffodil phytoene synthase gene into japonica rice, which led to the production of measurable amounts of phytoene in the rice endosperm (7). Although this was a great achievement, it was not sufficient to enable b-carotene synthesis. The next step was to insert a phytoene desaturase gene taken from the soil bacteria Erwinia uredovora (43). With this genetic transformation, success was achieved. These two foreign genes were able to turn on the carotenoid biosynthetic pathway, and up to 1.6 µg of total carotenoids/g could be synthesized, which turned the rice endosperm from white to a golden yellow (Fig. 3). At the beginning of the 21st century, amid much contention and debate, Golden Rice made headlines as the first transgenically biofortified food crop.
Since then, improvements to Golden Rice have been made by replacing the daffodil phytoene synthase gene with a maize phytoene synthase gene, allowing for accumulation of up to 37 µg of total carotenoids/g in Golden Rice 2, which provides up to 50% of the recommended daily allowance of vitamin A (30). Due to concerns over the safety of transgenic crops, Golden Rice has yet to be made available to farmers and consumers, but recent safety validations by the U.S. Food and Drug Administration, as well as by regulatory agencies in Canada and Australia and New Zealand, are encouraging steps toward its commercialization (17,20,22).
Orange Maize. Biofortification of maize took a different path. Maize is a staple crop for billions of people in developing countries, particularly Africa, Central America, and Mexico, providing up to 30% of their total caloric intake (16,26). Through germplasm characterization, considerable natural variation in carotenoid concentrations in maize has been identified, and biofortification efforts through breeding have been underway since the early 2000s (4,18,24,25,28). On average, yellow maize grown around the world contains 2 µg of b-carotene/g, but varieties have been found with concentrations of up to 13.6 µg/g. These high–b-carotene varieties were used to introgress high-carotenoid alleles into white maize varieties that contained no carotenoids but that had better agronomic traits, resulting in up to 13 µg of b-carotene/g. To increase concentrations even more, several genomics studies were conducted to identify genetic markers controlling carotenoid concentrations. Four major genetic markers in genes that encode lycopene e-cyclase, b-carotene hydroxylase, zeaxanthin epoxidase, and e-carotene hydroxylase were identified and subsequently used in marker-assisted selection, doubling the b-carotene concentrations up to 26 µg/g (3,19,29,42,45). These varieties were used to introgress the high–b-carotene alleles into locally adapted varieties grown in target countries.
Demonstrating the essentiality of market research in biofortification strategies, a study conducted in 2008 found that consumers in target countries for biofortified maize had a strong preference for white maize and considered yellow maize to be low-quality grain suitable for animal feed (10). Yellow biofortified maize, despite its potential benefits, would be met with little consumer acceptance. Orange maize, on the other hand, did not have negative associations, so to improve acceptance, scientists used their knowledge of the carotenoid biosynthetic pathway in maize to boost the orange colored carotenoids while maintaining high levels of b-carotene. In 2012, the first varieties of orange maize, containing 8 µg of b-carotene/g, were released in Zambia, which was followed by a second release in 2015 of improved varieties containing 10 µg of b-carotene/g. Currently, more than 40 varieties of orange maize are grown and consumed in 9 countries in Africa (1).
Red Sorghum. These rice and maize carotenoid biofortification efforts are also examples of highly successful international, multi-institutional, multidisciplinary initiatives that can serve as roadmaps for efforts in other crops and traits. Work in my lab has focused on using nutritional genomics to develop biofortification resources for health-promoting phytonutrients in sorghum. Sorghum is a dietary staple consumed by millions of people in sub-Saharan Africa and Asia (14). In the United States it is primarily used for animal feed; however, it is increasingly being used in high-value specialty foods marketed as “ancient grains,” “GMO-free,” “gluten-free,” or source of “antioxidants” (13,21,37). The antioxidant actions of sorghum are attributed to polyphenolic compounds found in the grain, particularly condensed tannins and 3-deoxyanthocyanidins. Consumption of polyphenols—a large group of phytochemicals commonly found in abundance in fruits—is correlated with lower incidence of chronic inflammatory diseases such as heart disease, cancer, and type 2 diabetes. Unlike many other cereals, sorghum retains a significant amount of polyphenol diversity and so has the potential to be used for breeding health-promoting specialty crops.
Most studies of sorghum have only explored the health benefits of a small number of sorghum varieties, but the U.S. Department of Agriculture (USDA) crop genebank maintains seed for more than 45,000 genetically distinct varieties of sorghum (38). For our research, we used the USDA genebank to select a panel of hundreds of genetically diverse varieties meant to capture all the genetic variation within sorghum and supplemented the panel with a subset of varieties that were known or suspected to contain polyphenols (8,33). We grew the varieties for three years in a row and measured their polyphenol concentrations. The results showed that our samples contained a large range of polyphenol concentrations, indicating that breeding could be an option for polyphenol biofortification in sorghum (32,33). To identify genetic markers controlling polyphenol variation among the varieties, we genotyped each variety in the panel and conducted genome-wide association studies. Several genetic markers were identified, including two markers near Tannin 1 and Tannin 2—the two major genes known to be involved in condensed tannin biosynthesis (41).
Next, to narrow the selection of high-polyphenol varieties to use for breeding and to characterize health benefits, we wanted to identify variation in anti-inflammatory properties. We extracted the polyphenols from a subset of genetically related varieties and tested them in a mouse macrophage cellular model of inflammation (34). Varieties that significantly decreased inflammatory proteins were identified and can be tested further in animal and human studies to determine their anti-inflammatory potential. Currently, high-polyphenol sorghum is used in a breakfast cereal line (Grain Berry Cereals) and sold through a sorghum specialty company (Nu Life Market). Newly discovered high-polyphenol varieties and genetic markers could be used to breed additional improved specialty varieties for this niche market.
Future Trends
Tremendous progress has been made in nutritional genomics over the last couple of decades, but more needs to be done to help alleviate malnutrition worldwide. As scientists and breeders increasingly incorporate nutrition-related goals into their research programs, we will see increasing numbers of biofortified crops tailored to specific environments, uses, and diseases. With more than 2.5 billion malnourished people in the world and a projected population of nearly 10 billion by 2050, we face a challenging road ahead of us in agriculture. Nutritional genomics research is helping to meet these challenges by accelerating biofortification through new discoveries in genetic diversity, molecular controls, and health benefits of nutrients in food crops and will be a key contributor to a food secure future.
Acknowledgments
This study was carried out using resources at the KSU Integrated Genomics Facility and Beocat High Performance Computing cluster. Support for this study was provided by the Kansas Grain Sorghum Commission and K-State Research and Extension. This study is contribution No. 19-076-J from the Kansas Agricultural Experiment Station.
Davina Rhodes is an assistant professor of nutritional genomics in the Department of Agronomy at Kansas State University. She has a master’s degree in human nutrition and a Ph.D. degree in integrative biology. Her research focuses on the nutritional improvement of food crops, particularly molecular breeding resources for increased carotenoids in sorghum grain.
References References
- Andersson, M. S., Saltzman, A., Virk, P. S., and Pfeiffer, W. H. Progress update: Crop development of biofortified staple food crops under HarvestPlus. Afr. J. Food Agric. Nutr. Dev. 17:11905, 2017.
- Araus, J. L., and Cairns, J. E. Field high-throughput phenotyping: The new crop breeding frontier. Trends Plant Sci. 19:52, 2014.
- Babu, R., Palacios, N., and Prasanna, B. Biofortified maize—A genetic avenue for nutritional security. Page 161 in: Translational Genomics for Crop Breeding: Abiotic Stress, Yield and Quality, vol. 2. R. K. Varshney and R. Tuberosa, eds. John Wiley & Sons Ltd., Chichester, U.K., 2013.
- Berardo, N., Mazzinelli, G., Valoti, P., Laganà, P., and Redaelli, R. Characterization of maize germplasm for the chemical composition of the grain. J. Agric. Food Chem. 57:2378, 2009.
- Bevan, M. W., Uauy, C., Wulff, B. H., Zhou, J., Krasileva, K., and Clark, M. D. Genomic innovation for crop improvement. Nature 543:346, 2017.
- Bouis, H. E., and Saltzman, A. Improving nutrition through biofortification: A review of evidence from HarvestPlus, 2003 through 2016. Glob. Food Sec. 12:49, 2017.
- Burkhardt, P. K., Beyer, P., Wünn, J., Klöti, A., Armstrong, G. A., Schledz, M., von Lintig, J., and Potrykus, I. Transgenic rice (Oryza sativa) endosperm expressing daffodil (Narcissus pseudonarcissus) phytoene synthase accumulates phytoene, a key intermediate of provitamin A biosynthesis. Plant J. 11:1071, 1997.
- Casa, A. M., Pressoir, G., Brown, P. J., Mitchell, S. E., Rooney, W. L., Tuinstra, M. R., Franks, C. D., and Kresovich, S. Community resources and strategies for association mapping in sorghum. Crop Sci. 48:30, 2008.
- Crossa, J., Pérez-Rodríguez, P., Cuevas, J., Montesinos-López, O., Jarquín, D., et al. Genomic selection in plant breeding: Methods, models, and perspectives. Trends Plant Sci. 22:961, 2017.
- De Groote, H., and Kimenju, S. C. Comparing consumer preferences for color and nutritional quality in maize: Application of a semi-double-bound logistic model on urban consumers in Kenya. Food Policy 33:362, 2008.
- DellaPenna, D. Nutritional genomics: Manipulating plant micronutrients to improve human health. Science 285:375, 1999.
- Development Initiatives. Global Nutrition Report 2017: Nourishing the SDGs. Available online at www.gainhealth.org/wp-content/uploads/2017/11/GNR-Report_2017.pdf. Development Initiatives, Bristol, U.K., 2017.
- Elbehri, A. The changing face of the U.S. grain system: Differentiation and identity preservation trends. Economic Research Report No. 35. Published online at www.ers.usda.gov/webdocs/publications/45729/11887_err35_1_.pdf?v=41056. U.S. Department of Agriculture, Economic Research Service, Washington, DC, 2007.
- Food and Agriculture Organization of the United Nations. Sorghum and Millets in Human Nutrition. Published online at www.fao.org/docrep/t0818e/T0818E00.htm. FAO, Rome, Italy, 1995.
- Food and Agriculture Organization of the United Nations. The Second Report on the State of the World’s Plant Genetic Resources for Food and Agriculture. Published online at www.fao.org/docrep/013/i1500e/i1500e.pdf. FAO, Rome, Italy, 2010.
- Food and Agriculture Organization of the United Nations. FAOSTAT: Food balance sheets. Available online at www.fao.org/faostat/en/#data/FBS. FAO, Rome, Italy, 2017.
- Food Standards Australia New Zealand. Application A1138 – Food derived from provitamin A rice line GR2E. Published online at www.foodstandards.gov.au/code/applications/Pages/A1138GMriceGR2E.aspx. FSANZ, Majura Park, Australia, and Wellington, New Zealand, 2017.
- Halilu, A. D., Ado, S. G., Aba, D. A., and Usman, I. S. Genetics of carotenoids for provitamin A biofortification in tropical-adapted maize. Crop J. 4:313, 2016.
- Harjes, C. E., Rocheford, T. R., Bai, L., Brutnell, T. P., Kandianis, C. B., et al. Natural genetic variation in lycopene epsilon cyclase tapped for maize biofortification. Science 319:330, 2008.
- Health Canada. Novel food information—Provitamin A biofortified rice event GR2E (Golden Rice). Published online at www.canada.ca/en/health-canada/services/food-nutrition/genetically-modified-foods-other-novel-foods/approved-products/novel-food-information-golden-rice-gr2e.html. Novel Food Section, Health Canada, Ottawa, ON, Canada, 2018.
- Janzen, E. L., and Wilson, W. W. Cooperative marketing in specialty grains and identity preserved grain markets. Agribusiness & Applied Economics Report 23558. North Dakota State University, Department of Agribusiness and Applied Economics, Fargo, ND, 2002.
- Keefe, D. Biotechnology consultations on food from GE plant varieties. BNF No. 158: Rice. Published online at www.accessdata.fda.gov/scripts/fdcc/?set=Biocon&id=IR-00GR2E-5. U.S. Food and Drug Administration, Silver Spring, MD, 2018.
- Li, H., Rasheed, A., Hickey, L. T., and He, Z. Fast-forwarding genetic gain. Trends Plant Sci. 23:184, 2018.
- Li, S., Tayie, F. A. K., Young, M. F., Rocheford, T., and White, W. S. Retention of provitamin A carotenoids in high β-carotene maize (Zea mays) during traditional African household processing. J. Agric. Food Chem. 55:10744, 2007.
- Menkir, A., Liu, W., White, W. S., Maziya-Dixon, B., and Rocheford, T. Carotenoid diversity in tropical-adapted yellow maize inbred lines. Food Chem. 109:521, 2008.
- Menkir, A., Palacios-Rojas, N., Alamu, O., Dias Paes, M. C., Dhiwayo, T., et al. Vitamin A-biofortified maize: Exploiting native genetic variation for nutrient enrichment. Published online at www.harvestplus.org/sites/default/files/publications/Vitamin-A-Science-Brief.pdf. Science Brief Biofortification, No. 2, February. CIMMYT, IITA, EMBRAPA, HarvestPlus, and Crop Trust, Bonn, Germany, 2018.
- Morris, G. P., Ramu, P., Deshpande, S. P., Hash, C. T., Shah, T., et al. Population genomic and genome-wide association studies of agroclimatic traits in sorghum. Proc. Natl. Acad. Sci. U.S.A. 110:453, 2013.
- Ortiz-Monasterio, J. I., Palacios-Rojas, N., Meng, E., Pixley, K., Trethowan, R., and Peña, R. J. Enhancing the mineral and vitamin content of wheat and maize through plant breeding. J. Cereal Sci. 46:293, 2007.
- Owens, B. F., Lipka, A. E., Magallanes-Lundback, M., Tiede, T., Diepenbrock, C. H., et al. A foundation for provitamin A biofortification of maize: Genome-wide association and genomic prediction models of carotenoid levels. Genetics 198:1699, 2014.
- Paine, J. A., Shipton, C. A., Chaggar, S., Howells, R. M., Kennedy, M. J., et al. Improving the nutritional value of Golden Rice through increased pro-vitamin A content. Nat. Biotechnol. 23:482, 2005.
- Potrykus, I. From the concept of totipotency to biofortified cereals. Annu. Rev. Plant Biol. 66:1, 2015.
- Rhodes, D., Gadgil, P., Perumal, R., Tesso, T., and Herald, T. J. Natural variation and genome-wide association study of antioxidants in a diverse sorghum collection. Cereal Chem. 94:190, 2017.
- Rhodes, D., Hoffmann, L., Rooney, W. L., Ramu, P., Morris, G. P., and Kresovich, S. Genome-wide association study of grain polyphenol concentrations in global sorghum [Sorghum bicolor (L.) Moench] germplasm. J. Agric. Food Chem. 62:10916, 2014.
- Rhodes, D., and Kresovich, S. Sorghum [Sorghum bicolor (L.) Moench] genotypes with contrasting polyphenol compositions differentially modulate inflammatory cytokines in mouse macrophages. J. Chem. DOI: http://dx.doi.org/10.1155/2016/9640869. 2016.
- Ricroch, A. E. Assessment of GE food safety using ‘-omics’ techniques and long-term animal feeding studies. New Biotechnol. 30:349, 2013.
- Taylor, J., Taylor, J. R. N., and Kini, F. Cereal biofortification: Strategies, challenges, and benefits. Cereal Foods World 57:165, 2012.
- Taylor, J. R. N., Schober, T. J., and Bean, S. R. Novel food and non-food uses for sorghum and millets. J. Cereal Sci. 44:252, 2006.
- U.S. Department of Agriculture, Agricultural Research Service. National Genetic Resources Program: Germplasm Resources Information Network. Available online at www.ars-grin.gov. National Germplasm Resources Laboratory, Beltsville, MD, 2015.
- von Grebmer, K., Saltzman, A., Birol, E., Wiesmann, D., Prasai, N., Yin, S., Yohannes, Y., Menon, P., Thompson, J., and Sonntag, A. 2014 Global hunger index: The challenge of hidden hunger. Welthungerhilfe, Bonn, Germany; International Food Policy Research Institute, Washington, DC; and Concern Worldwide, Dublin, Ireland. DOI: http://dx.doi.org/10.2499/9780896299580. 2014.
- World Health Organization. Global prevalence of vitamin A deficiency in populations at risk 1995–2005: WHO global database on vitamin A deficiency. Published online at http://apps.who.int/iris/bitstream/handle/10665/44110/9789241598019_eng.pdf;
jsessionid=D501FB75010A01321ED440DECE6E2E00?sequence=1. WHO, Geneva, Switzerland, 2009.
- Wu, Y., Li, X., Xiang, W., Zhu, C., Lin, Z., et al. Presence of tannins in sorghum grains is conditioned by different natural alleles of Tannin1. Proc. Natl. Acad. Sci. U.S.A. 109:10281, 2012.
- Yan, J., Kandianis, C. B., Harjes, C. E., Bai, L., Kim, E. H., et al. Rare genetic variation at Zea mays crtRB1 increases b-carotene in maize grain. Nat. Genet. 42:322, 2010.
- Ye, X., Al-Babili, S., Klöti, A., Zhang, J., Lucca, P., Beyer, P., and Potrykus, I. Engineering the provitamin A b-carotene) biosynthetic pathway into (carotenoid-free) rice endosperm. Science 287:303, 2000.
- Yu, X., Li, X., Guo, T., Zhu, C., Wu, Y., et al. Genomic prediction contributing to a promising global strategy to turbocharge gene banks. Nat. Plants 2:16150, 2016.
- Zunjare, R. U., Hossain, F., Muthusamy, V., Baveja, A., Chauhan, H. S., et al. Development of biofortified maize hybrids through marker-assisted stacking of b-Carotene Hydroxylase, Lycopene-e-Cyclase and Opaque2 genes. Front Plant Sci. 9:178, 2018.
|